4 Discussion
The findings in this study show that landing style (precision or running style landing) and movement choice (drop, step vault, dash vault, or kong vault) affect GRFs for parkour vaults. Landing style was found to have the greatest effect on vGRF, while movement choice had the greatest effect on bGRF. All movements significantly increased in vGRF when using a running landing. The kong vault was found to produce the greatest vGRF and bGRF, differing significantly from all other movements in bGRF when using a running landing, and in both vGRF and bGRF for precision landings. The dash vault produced the least vGRF of all movements in both landing styles, but only significantly differed from the drop and kong vault in vGRF when using a running landing. The dash also produced significantly lower bGRF than any other movement when using a running landing. The step vault was not found to differ significantly from any other movement except the kong vault in vGRF and, in particular, did not significantly differ from the drop in any dependent variable with any landing style.
4.1 Effects of landing style
vGRFs for precision style drop landings were 1.57 x BW per leg in this study. These values are in line with those found by Puddle and Maulder (2013) (3.2 / 2 = 1.6 x BW per leg) and Standing and Maulder (2015) (3.6 / 2 = 1.8 x BW per leg) for drop landings by traceurs. Puddle and Maulder (2013) used a fixed platform height of 0.75 m while Standing and Maulder (2015) used a maximum drop height of 50% participant height, which the obstacle used in the present study exceeded for all participants. Yeow, Lee and Goh (2009) modelled vertical ground reaction force and drop height as a regression relationship, proposing that vGRF increases exponentially as drop height increased. This relationship has so far not been demonstrated for traceurs. Drop landing vGRFs remain similar among these studies despite height increases. However, participants in the present study were instructed to crouch fully before dropping, as opposed to standing upright and stepping off as they were in Puddle and Maulder (2013) and Standing and Maulder (2015). As such, the true drop heights between studies may not differ greatly if participant centre of mass is considered rather than platform height. Even so, the present study results are lower than those previously reported, despite participants not being explicitly instructed to land as softly as possible as they were in prior parkour studies. This may indicate that efforts to minimise GRFs in precision landings are habitual and automatic in experienced traceurs, adopted as a default landing strategy.
While peak vGRF increased for running style drop landings, the peak vGRF found in this study remains lower (3.21 x BW) than those found in a study on single foot landings by figure skaters (3.5 x BW), which used a much lower platform height of only 0.2 m (Saunders et al., 2014). Yeow, Lee and Goh (2011) also found larger peak vGRFs in single-leg drop landings (4+ x BW) performed by recreational athletes with a platform height of 0.6 m. If no mitigating techniques for single-leg landings were being utilised by traceurs, it could be expected to see similar or larger vGRFs with the increase in platform height in this study. It appears that traceurs are applying techniques to minimise vGRF in single-leg landings, in similarity to the previously established findings of reduced vGRF in two-legged drop landings performed by traceurs.
The knee is the primary dissipator of energy in precision landings (Maldonado, Soueres and Watier, 2018). The knee has been shown to respond less effectively to single-leg landings than double-leg landings in drop jumps (Yeow, Lee and Goh, 2010) and therefore higher vGRF due to the lack of knee flexion in a running style landing, regardless of movement choice, could be expected. While vGRFs did increase in running landings in this study, they were still lower than vGRFs reported for single-leg landings by other athletes. The plantar flexors of the calf have been shown to be effective at absorbing impact through the ankle joint when knee flexion is restricted, possibly even more so than when the knee can freely flex (Self and Paine, 2001). The lack of knee flexion may instead shift responsibility onto the other joints of the lower limb to absorb vGRF. As such, it may not solely be the long duration of eccentric loading of the quadriceps and hip muscles that contributes to reduced vGRFs in traceur landings, but also contributions from an effectively engaged calf complex due to a raised heel.
It should be noted that cited studies utilised a stop landing on a single-leg, whereas the present study utilised a running style single-leg landing. Yeow, Lee and Goh (2011) also found that frontal plane energetics increased in single-legged stop landing tasks due to the increased demand for stability, which could not be as effectively dissipated as sagittal energetics due to the lack of range of motion in the knee joint in that plane. It may be that continued motion when landing on one leg decreases the need to seek stability compared to a stop landing, similar to how increased speed requires less rider input to keep a bicycle upright (Sharp, 2008). Future research may consider the effect of various speeds when exiting a vault and the effect on GRF, particularly in running style landings.
4.2 Parkour vault characteristics
The kong vault resulted in significantly greater vGRFs and bGRFs than all other movements with a precision landing, and even in comparisons that did not achieve significance, always produced at least a medium to large effect size. This may be due to the need for an increase in height of the traceur’s centre of mass to allow space for the knees and feet to pass over the obstacle, increasing the height of the drop on the landing side. Yet, the increase in vGRF is greater than could be expected for the relatively small height gain needed to clear the obstacle with a kong vault, given the similarity between vGRFs for drop landings from different heights discussed above. The diving nature of the movement may also increase horizontal speed, with a resultant increase in bGRF on landing. This diving execution also places the traceur in a flexed hip position once over the obstacle, with a more anteriorly tilted trunk. Trunk flexion is an important contributor to mitigating landing GRFs (Blackburn and Padua, 2008) and, as drop heights increase, the role of proximal joints in energy dissipation also increases (Nordin and Dufek, 2017). Reduced hip flexion range due to an already anteriorly tilted trunk when landing may result in a decreased ability for the hip to contribute to energy dissipation and result in greater GRFs. Further, vGRF for the kong was not significantly greater than a drop landing when using a running landing style. The need to stretch the landing leg towards the ground and raise the trunk into a running stance would extend the hip joint, differentiating it from a kong performed with a precision landing. However the role of trunk position in landing is still being investigated, with an overly upright trunk on landing shown to increase knee valgus angles and the risk of ACL injury (Saito et al., 2020). The role of trunk position in PKVs could be further studied through kinematic 2D or 3D motion tracking of traceurs paired with kinetic GRF analysis.
Although consistently producing the least GRFs of any movement, performance of the dash vault varied between the two landing styles. Dash vaults with precision landings did not differ from the drop or step vault in vGRF; however in running style landings, the dash vault was found to produce significantly lower vGRFs than the drop landing and kong vault and lower bGRFs than all other movements. This may be due to the differing levels of support provided by the hands on the obstacle as the traceur descends to land. In a running dash vault, the leading leg reaches the floor before the hands leave the obstacle, while in a precision landing both hands leave the obstacle before landing occurs. Further, due to the sole use of the hands on the obstacle without a concurrent trailing lower limb, this ability to reach for the floor before the supporting limbs leave the obstacle is maintained for a greater height than the step vault. This may indicate that the longer a traceur can maintain support on the obstacle behind them, the greater the contribution of the upper limbs to reductions in vGRF. In turn, this may increase the risk of wrist injury in the dash vault, with GRFs through the hands in some high-intensity gymnastic vaults found to exceed 1.3 x BW (Penitente and Sands, 2015). Further research on the forces experienced in the upper limb during PKVs may reveal more about this relationship and provide insight on potential upper limb injury risks inherent to PKVs.
The step vault did not significantly differ from a drop of equivalent height in peak vGRF or bGRF for either landing style, although there was still a moderate effect on the reduction of peak vGRF with the step vault when using a running landing. The height of the obstacle used in these trials may have affected this outcome. The step vault technique requires reaching down to the ground while one foot and hand remains behind the traceur on the obstacle. If the obstacle is sufficiently low enough that the reaching foot can contact the floor without the trailing limbs leaving the obstacle, then more support may be provided to the landing foot. This was not possible for all participants due to height differences, with the trailing limbs leaving the obstacle before contact was made with the floor. Execution of the step vault in this study also tried to simulate typical use of a step vault as part of a route, resulting in performance at speed. Moderating the speed a movement is performed can increase control of that movement (Frost et al., 2015). Moderation of speed of execution, with an effort to more deliberately lower the leading foot to the floor with prolonged support of the hand and foot on the obstacle, could result in lower GRFs for the step vault. Nonetheless, the step vault is often taught as a beginner vault, and so this finding may be of importance in determining the risk of injury for new practitioners. As such, the step vault should not be considered the absolute safest or least impactful of the PKVs by default. Due care and attention to obstacle height and speed of execution should be considered when teaching the step vault to beginners to mitigate the risk of exposing them to larger GRFs before they are physically prepared for them.
4.3 Implications for injury risk
The results of this study show that, despite still displaying an ability to mitigate landing forces when landing on a single limb, a traceur does increase GRFs when utilising a running landing compared to a precision landing. Yet, vGRF figures reported in this study are lower than those reported in traditional flat running of around 3 x BW (Trowell et al., 2019) for the step and dash vault and only slightly above it for the kong vault. Such levels of GRF are considered unlikely to cause acute injury through impact alone (Kuntze, Sellers and Mansfield, 2009). This is in agreement with a survey by Wanke et al. (2013) whereby traceurs reported that most acute parkour injuries occurred due to misjudgement or overestimation rather than impact trauma in an otherwise ideal landing scenario. Consequently, the overall risk of acute injury to the lower limb with single-leg running style landings likely remains low for experienced traceurs.
However, chronic injury remains a potential risk for traceurs of all levels. Frequent exposure to the vGRF and bGRF levels found in this study for both precision and running style landings could place an athlete at risk of chronic injury (Hreljac, 2004). To that end, the GRF results obtained in this study have allowed the development of a rudimentary intensity index between all four movements across both landing styles, demonstrated in Figure 4.1. A drop precision landing is used as a baseline intensity of 1 for both vGRF and bGRF, with all other movements standardised to this same scale using the formulas
Parkour is a developing sport. Practitioners and coaches rely mostly on personal subjective experience to effectively determine the appropriate intensity of training. An intensity index could instead be used as a tool in the field to objectively control training intensity alongside traditional training parameters such as volume and frequency (Wallace et al., 2010). Given the limitations and scope of the present study, this index is presented as an example only. Further development of an index utilising such things as different heights, speeds, landing distances, and a wider subject pool may further aid in developing it as a useful tool for field use.
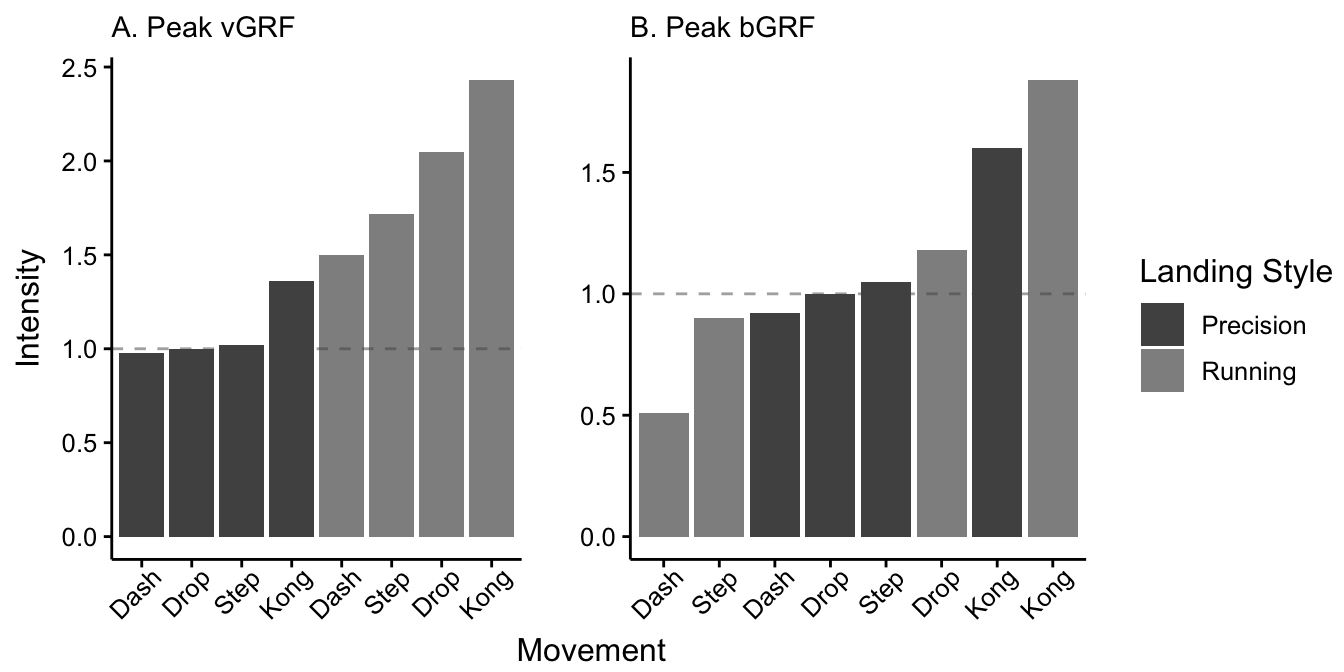
Figure 4.1: Intensity plots for peak vertical (A) and braking (B) ground reaction forces, standardised with the drop precision landing as an intensity of 1.
4.4 Limitations
As can be seen in Figure 3.1, ICCs for movement and landing style combinations varied; while most achieved at least a ‘moderate’ reliability, confidence intervals were broad for all groups. Visual analysis of the force profiles for the three trials of each combination for each participant seemed to show a trend where, if the peak values were not evenly spaced, one trial would be noticeably above or below the other two. It appears that vaulting movements may be highly variable in execution, even amongst experienced practitioners, although some limitations in this study may also have affected this result. While practitioners were instructed to keep run-up distances the same for each movement repetition, this was not strictly measured and standardised. Variations in run-up distance may have contributed to intra-participant variation between trials. Participants also attended in their own footwear, which may have contributed to inter-participant differences due to differing levels of cushioning affecting the amount of GRFs absorbed by the sole (Cheung and Ng, 2008).
One phenomenon of the data noted on examination of the force curves is the presence of multiple force peaks in the vertical axis. Multiple vGRF peaks in a drop landing are common, with the first peak commonly representing toe contact, the second peak for heel contact, and the third peak for muscle activation slowing descent (Bauer et al., 2001). Usually the second peak is the largest, but in the present study this was not always the case, with the first peak sometimes exceeding the second. This may be due to the lack of heel contact during a precision technique landing. The present study did not distinguish between maximum vGRF occurring at the first or second peak. As a result, the maximum vGRF for some movements and landing styles was taken from the first peak and some from the second. Future studies may wish to isolate the peaks to determine how each contributes to the energy dissipation of a landing. In particular, if the goal is to analyse the rate of force development (RFD) in these movements, careful analysis of the patterns of peaks presenting should be considered to ensure correct RFD calculations.
Single limb GRFs were estimated for the precision landing style by halving the measurements obtained from both legs on the force platform, with the assumption that participants have no bilateral asymmetries in their lower limbs. While measuring GRFs with two feet and halving the figure is not expected to give an exact figure for the peak GRF of a single limb due to small timing difference between limbs, it is shown to produce results close to modelled figures for single-legged landings (Niu et al., 2014). Two-legged landings in the precision style usually involve keeping the ankles close together; splitting the feet across two force plates, while potentially improving single limb GRF measurement accuracy, may result in altered landing mechanics and a loss of validity. Nonetheless the figures obtained for the force per single limb in precision landings used in this study should be considered with this possible degree of imprecision in mind.
Ensuring ecological and external validity (Pinder et al., 2011) when studying parkour movement is difficult due to the inherent free-form nature of the sport and the many different environments in which it is practised. This study has attempted to examine some of the most commonly observed parkour vaults and landing styles, but a wide variety remains. Precise execution of the same technique may vary between traceurs, determined by their training history and practice rather than some agreed-upon, centrally-defined, method. Only 10 traceurs participated in this study, many of whom had previously trained together. Traceurs from other social groups, locales, and demographics may have developed different approaches to techniques and their execution with different landing styles.
There is currently no widely accepted standardisation of parkour movement, either in the form of a rulebook for a competitive event, guidelines from a governing body, or even in some cases, a common consensus among traceurs. Therefore in order to study parkour, some constraints have to be selected by researchers and placed upon the wide variety of parkour movements available for a given task. It is important to the nature of the sport that these efforts to constrain movements for study are purely descriptive, and not intended to prescribe or restrict parkour movement in any fashion beyond the scope of the study being undertaken.
The obstacle height and distance from the force plate may have artificially constrained the performance of some techniques, with some participants describing the landing point as being too close for regular execution of some movements and some landing styles. In some ways this may increase ecological validity for the study, as when performing parkour outside there is a similar inability to adjust your choice of landing distance - a traceur cannot move a brick wall or a steel railing forward to be more accommodating. But a traceur’s movement choice is dictated not just by preference, but by the environment and their reaction to it (Croft and Bertram, 2017). A certain technique may better suit a desired landing distance. Such difference may be dictated by the projectile path that the participant takes over the obstacle, similar to changing the projectile arc for a ball to better hit a target in a certain plane. Future studies on the projectile motion of traceurs during the execution of vaults to different landing distances could investigate the task-specific suitability of individual vaults.